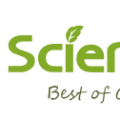
he emergence of RNA synthesis in vitro and in vivo delivery methods has garnered significant attention in the biomedical field, particularly regarding the applications of synthetic mRNA. However, in most biological systems, the half-life of exogenous mRNA is limited, especially in rapidly dividing cells. In contrast, viral RNA can extend its lifespan by actively replicating within the host. Consequently, they can serve as a framework for designing synthetic self-replicating RNA (srRNA), which can be used to increase the half-life and intracellular concentration of the encoded RNA. Synthetic srRNA can be employed to enhance the expression of recombinant proteins or induce cellular reprogramming into pluripotent stem cells. This discussion will provide detailed insights into the design principles of srRNA, its viral origins, current challenges, and its applications in areas such as mRNA vaccines and stem cell reprogramming.
Directions for srRNA Applications
1. Self-replicating RNA Vaccines
With the emergence of the COVID-19 pandemic, mRNA vaccines entered clinical trials at the forefront, and the first batch of volunteers received vaccinations within 10 weeks of the release of the SARS-CoV-2 genetic sequence. The saRNA constructs used for vaccine applications are derived from alphaviruses, such as Venezuelan equine encephalitis virus (VEEV) or Semliki Forest virus (SFV). These saRNA constructs contain four non-structural proteins, a subgenomic promoter, and the target gene (replacing viral structural proteins). By deleting the viral structural proteins, the RNA cannot produce infectious virus. Once delivered into the cytoplasm, the non-structural proteins form an RNA-dependent RNA polymerase (RDRP), which replicates the entire RNA chain. Each of the four non-structural proteins plays a role in the formation of RDRP, which is a complex multi-stage process. This RNA replication results in higher antigen expression compared to non-replicating mRNA, providing the ability for amplification and sustained antigen production in the body.
2. Stem Cell Research
Induced pluripotent stem cells (iPSCs) are among the most promising cells in regenerative medicine because they can proliferate indefinitely and differentiate into any type of cell lost due to disease or injury within the body. The formation of iPSCs can be achieved through the induction of “reprogramming” genes such as Myc, Oct3/4, Sox2, and Klf4. Typically, these four genes are introduced through retroviral or lentiviral systems, integrating the desired genes into the host genome. This method of cellular reprogramming into iPSCs carries the risk of inserting mutations into the target cell genome, which is disadvantageous for the cells and may even lead to tumorigenesis. Utilizing mRNA for non-integrating genome editing is an attractive alternative for cell reprogramming. However, this approach is often hindered by the limited half-life of mRNA, requiring daily transfections. srRNA can induce pluripotency more efficiently, making it more suitable for potential therapeutic applications. For example, cell reprogramming can be achieved using RNA vectors derived from Sendai virus (SeV), which have been shown to be incapable of infectious reproduction. Studies have demonstrated that both mouse and human fibroblasts can encode the four Yamanaka reprogramming factors after SeV infection for 14 days. Similarly, srRNA based on VEEV can successfully reprogram adult fibroblasts and kidney epithelial cells into iPSCs.
Conditions for RNA Self-Replication
1. Natural Self-Replicating RNA
srRNA holds the potential to serve as an alternative to traditional genome engineering methods that do not integrate into the genome. However, research into which RNA species can be used to generate functional srRNA is still in its early stages. To understand which RNA systems can be transformed into synthetic RNA replicons, it is necessary to comprehend the mechanisms of RNA capable of self-replication in vivo or in vitro.
It is speculated that the largest group of self-replicating RNA in the natural world consists of RNA viruses and viroids. According to the Baltimore virus classification system, RNA viruses without a DNA intermediate can be divided into three groups (III, IV, V), depending on the overall structure and polarity of their genomes. Therefore, Group III consists of all double-stranded RNA viruses (dsRNA), Group IV and Group V consist of single-stranded RNA viruses (ssRNA) with positive (+) and negative (−) genome polarity, respectively. In the case of +ssRNA viruses, the genome RNA must at least partially overlap with the mRNA needed for gene expression, meaning it includes coding sequences. In the case of −ssRNA viruses, the genome must first be transcribed to produce the complementary (+) strand that contains the coding sequences. Thus, the virus RdRP is an essential component of the −ssRNA virus particle. The dsRNA virus genome consists of (+) and (−) strand RNAs, which are packaged together with the virus RdRP within the virus capsid. In some cases, the complete genome of an RNA virus is not encoded on a single RNA chain but can be divided into several segments, similar to how genome information is distributed between chromosomes. Importantly, the (+) strand version of the virus genome is not necessarily the only mRNA that is translated. In several RNA viruses, some of the encoded genes are translated from subgenomic transcripts transcribed from the genome’s (−) strand using internal or subgenomic promoters.
It’s worth noting that recently discovered double-stranded viruses (ssRNA viruses with coding sequences on both strands) and some segmented ssRNA viruses with double-stranded genomes (combinations of segments with positive or negative polarity) do not belong to either Group IV or Group V but have formed a separate classification. Additionally, in riboviruses, viroids, and potentially some double-stranded viruses, the ssRNA genome can be circularized, thereby protecting the RNA ends from the action of exonucleases.
Regardless of classification, the complete replication of all these RNA species requires the synthesis of both (+) and (−) strands. In linear +ssRNA and −ssRNA viruses, replication of the genome strand begins with the synthesis of the complementary strand using the genome strand as a template. In the case of dsRNA viruses, replication of the genome dsRNA typically starts with the synthesis of the (+) strand, which is also used as mRNA for virus gene expression. Then, after this step, the newly synthesized (+) strand is used as a template to synthesize the second strand.
Typically, the replication of circular RNA in riboviruses, viroids, and potential double-stranded RNA viruses is based on rolling-circle replication. Circular genomic RNA serves as a template for the continuous synthesis of linear repeated sequences of antigenomic strands. These intermediates are subsequently processed into monomeric intermediates, which are then circularized into circular antigenomic RNA. Circular antigenomic RNAs can then serve as templates for the synthesis of new genomic circular RNA, similar to how they were originally generated. Alternatively, in the hybridization between linear and circular RNA replication, the connected linear intermediates have already been used as templates to synthesize the corresponding complementary strands. Typically, the processing of linear intermediates into circular products requires the action of co-encoded ribozymes or host factors.
2. Autonomous Replication and Coding Potential of srRNA
RNA viruses, including viroids, are intracellular parasites and, therefore, rely on host factors to complete their life cycle. However, the degree of autonomy in RNA self-replication can vary significantly. This distinction sets apart “active” srRNA, which carries a dedicated RdRP gene, from passive replicating RNA that lacks self-encoded polymerases. As a result, active srRNA may have a broader host range compared to passive replicating RNA.
Viroids and satellite RNA viruses (including virophages) are purely passive replicators as they do not encode any RNA polymerase themselves. Therefore, they rely entirely on host polymerases or co-infecting helper viruses’ polymerases to amplify their genomic RNA. In contrast to these simple RNA pathogens, all non-satellite RNA viruses encode at least RdRP enzyme subunits, and as genome size increases, the number of encoded RdRPs also increases, thus enhancing the autonomy of RNA replication. Larger RNA viruses, such as VEEV, encode complete RdRPs in their genomes. These larger RNA viruses are less dependent on the host since many of them also encode additional RNA modification factors involved in processes like 5’ Cap and 3’ polyA, which are required for translation and potential RNA replication.
Source: https://www.bocsci.com/blog/principles-and-applications-of-self-replicating-rna-design/
With the emergence of the COVID-19 pandemic, mRNA vaccines entered clinical trials at the forefront, and the first batch of volunteers received vaccinations within 10 weeks of the release of the SARS-CoV-2 genetic sequence. The saRNA constructs used for vaccine applications are derived from alphaviruses, such as Venezuelan equine encephalitis virus (VEEV) or Semliki Forest virus (SFV). These saRNA constructs contain four non-structural proteins, a subgenomic promoter, and the target gene (replacing viral structural proteins). By deleting the viral structural proteins, the RNA cannot produce infectious virus. Once delivered into the cytoplasm, the non-structural proteins form an RNA-dependent RNA polymerase (RDRP), which replicates the entire RNA chain. Each of the four non-structural proteins plays a role in the formation of RDRP, which is a complex multi-stage process. This RNA replication results in higher antigen expression compared to non-replicating mRNA, providing the ability for amplification and sustained antigen production in the body.
2. Stem Cell Research
Induced pluripotent stem cells (iPSCs) are among the most promising cells in regenerative medicine because they can proliferate indefinitely and differentiate into any type of cell lost due to disease or injury within the body. The formation of iPSCs can be achieved through the induction of “reprogramming” genes such as Myc, Oct3/4, Sox2, and Klf4. Typically, these four genes are introduced through retroviral or lentiviral systems, integrating the desired genes into the host genome. This method of cellular reprogramming into iPSCs carries the risk of inserting mutations into the target cell genome, which is disadvantageous for the cells and may even lead to tumorigenesis. Utilizing mRNA for non-integrating genome editing is an attractive alternative for cell reprogramming. However, this approach is often hindered by the limited half-life of mRNA, requiring daily transfections. srRNA can induce pluripotency more efficiently, making it more suitable for potential therapeutic applications. For example, cell reprogramming can be achieved using RNA vectors derived from Sendai virus (SeV), which have been shown to be incapable of infectious reproduction. Studies have demonstrated that both mouse and human fibroblasts can encode the four Yamanaka reprogramming factors after SeV infection for 14 days. Similarly, srRNA based on VEEV can successfully reprogram adult fibroblasts and kidney epithelial cells into iPSCs.
Conditions for RNA Self-Replication
1. Natural Self-Replicating RNA
srRNA holds the potential to serve as an alternative to traditional genome engineering methods that do not integrate into the genome. However, research into which RNA species can be used to generate functional srRNA is still in its early stages. To understand which RNA systems can be transformed into synthetic RNA replicons, it is necessary to comprehend the mechanisms of RNA capable of self-replication in vivo or in vitro.
It is speculated that the largest group of self-replicating RNA in the natural world consists of RNA viruses and viroids. According to the Baltimore virus classification system, RNA viruses without a DNA intermediate can be divided into three groups (III, IV, V), depending on the overall structure and polarity of their genomes. Therefore, Group III consists of all double-stranded RNA viruses (dsRNA), Group IV and Group V consist of single-stranded RNA viruses (ssRNA) with positive (+) and negative (−) genome polarity, respectively. In the case of +ssRNA viruses, the genome RNA must at least partially overlap with the mRNA needed for gene expression, meaning it includes coding sequences. In the case of −ssRNA viruses, the genome must first be transcribed to produce the complementary (+) strand that contains the coding sequences. Thus, the virus RdRP is an essential component of the −ssRNA virus particle. The dsRNA virus genome consists of (+) and (−) strand RNAs, which are packaged together with the virus RdRP within the virus capsid. In some cases, the complete genome of an RNA virus is not encoded on a single RNA chain but can be divided into several segments, similar to how genome information is distributed between chromosomes. Importantly, the (+) strand version of the virus genome is not necessarily the only mRNA that is translated. In several RNA viruses, some of the encoded genes are translated from subgenomic transcripts transcribed from the genome’s (−) strand using internal or subgenomic promoters.
It’s worth noting that recently discovered double-stranded viruses (ssRNA viruses with coding sequences on both strands) and some segmented ssRNA viruses with double-stranded genomes (combinations of segments with positive or negative polarity) do not belong to either Group IV or Group V but have formed a separate classification. Additionally, in riboviruses, viroids, and potentially some double-stranded viruses, the ssRNA genome can be circularized, thereby protecting the RNA ends from the action of exonucleases.
Regardless of classification, the complete replication of all these RNA species requires the synthesis of both (+) and (−) strands. In linear +ssRNA and −ssRNA viruses, replication of the genome strand begins with the synthesis of the complementary strand using the genome strand as a template. In the case of dsRNA viruses, replication of the genome dsRNA typically starts with the synthesis of the (+) strand, which is also used as mRNA for virus gene expression. Then, after this step, the newly synthesized (+) strand is used as a template to synthesize the second strand.
Typically, the replication of circular RNA in riboviruses, viroids, and potential double-stranded RNA viruses is based on rolling-circle replication. Circular genomic RNA serves as a template for the continuous synthesis of linear repeated sequences of antigenomic strands. These intermediates are subsequently processed into monomeric intermediates, which are then circularized into circular antigenomic RNA. Circular antigenomic RNAs can then serve as templates for the synthesis of new genomic circular RNA, similar to how they were originally generated. Alternatively, in the hybridization between linear and circular RNA replication, the connected linear intermediates have already been used as templates to synthesize the corresponding complementary strands. Typically, the processing of linear intermediates into circular products requires the action of co-encoded ribozymes or host factors.
2. Autonomous Replication and Coding Potential of srRNA
RNA viruses, including viroids, are intracellular parasites and, therefore, rely on host factors to complete their life cycle. However, the degree of autonomy in RNA self-replication can vary significantly. This distinction sets apart “active” srRNA, which carries a dedicated RdRP gene, from passive replicating RNA that lacks self-encoded polymerases. As a result, active srRNA may have a broader host range compared to passive replicating RNA.
Viroids and satellite RNA viruses (including virophages) are purely passive replicators as they do not encode any RNA polymerase themselves. Therefore, they rely entirely on host polymerases or co-infecting helper viruses’ polymerases to amplify their genomic RNA. In contrast to these simple RNA pathogens, all non-satellite RNA viruses encode at least RdRP enzyme subunits, and as genome size increases, the number of encoded RdRPs also increases, thus enhancing the autonomy of RNA replication. Larger RNA viruses, such as VEEV, encode complete RdRPs in their genomes. These larger RNA viruses are less dependent on the host since many of them also encode additional RNA modification factors involved in processes like 5’ Cap and 3’ polyA, which are required for translation and potential RNA replication.
Source: https://www.bocsci.com/blog/principles-and-applications-of-self-replicating-rna-design/
Comments
No comments yet :( Be the first to comment on this post!